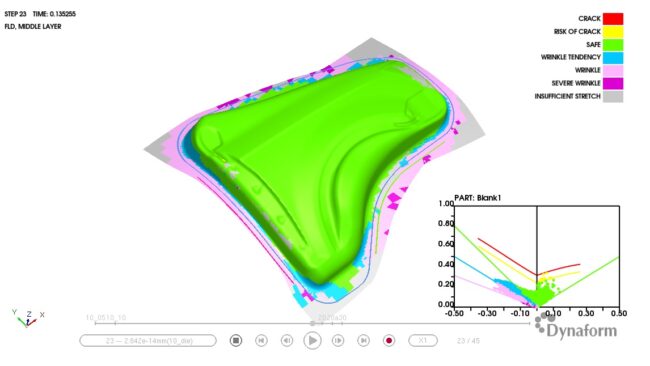
Sheet metal forming simulations play a pivotal role in modern manufacturing processes, enabling engineers to predict and optimise the behaviour of metal sheets during shaping operations. Whether it’s automotive panels, aircraft components, or consumer appliances, accurate simulations guide design decisions, reduce trial-and-error iterations, and enhance product quality.
By – Akshay Kharate
Let’s look into the critical factors that contribute to successful sheet metal forming simulations. From material modeling to boundary conditions, we explore the intricacies that engineers must address to achieve reliable results. Whether you’re a seasoned simulation expert or just stepping into the world of virtual forming, understanding these considerations is essential.
Material Behaviour Model:
Accurate material behaviour models utilise detailed stress-strain curves to capture a material’s response to applied loads, illustrating both elastic and plastic deformation phases. This representation is crucial for predicting how a material will perform under various loading conditions. Isotropic hardening models extend this understanding by describing how materials uniformly strengthen in all directions as they deform. This approach simplifies the analysis while still providing a reasonable approximation of material behaviour during repeated loading and unloading cycles, which is common in forming operations.
For even more precise simulations, anisotropic behaviour models are employed to account for direction-dependent properties of materials. Metals and composites, for instance, often exhibit different responses based on their internal structure and the direction of applied load. Anisotropic models consider variations in yield strength, stiffness, and hardening rates depending on orientation, providing a more accurate prediction of material behaviour during forming processes.
Mesh Quality:
A well-structured mesh ensures that the simulation can capture the necessary details of the physical phenomenon being modeled. Proper element size is a key aspect of mesh quality, especially near critical regions such as bends, flanges, and fillets where stress concentrations and complex deformations are likely to occur. In these areas, smaller elements are typically required to accurately resolve the gradients and intricate features, ensuring that the simulation can predict the behaviour of the material and structure under various loading conditions.
Avoiding excessive element distortion is equally important in maintaining mesh quality. Distorted elements can lead to numerical inaccuracies and convergence issues during the simulation. Therefore, it is essential to use elements that maintain a reasonable aspect ratio and are well-shaped throughout the mesh. Techniques such as mesh refinement, adaptive meshing, and the use of higher-order elements can help manage distortion and improve the overall quality of the mesh.
Boundary Conditions:
This includes specifying contact interactions between the tooling and the sheet metal, where accurate friction coefficients are crucial to model the resistance to sliding accurately. Initial gaps between the tool and the material must also be considered to ensure the simulation reflects real-world conditions. Properly defined boundary conditions help in predicting the material’s behaviour more accurately, leading to reliable and useful simulation results.
Tooling and Die Geometry:
Detailed representations of features such as draw beads, blank holders, and die radii, all of which play significant roles in controlling material flow and deformation comes under tooling and die geometry. Draw beads help manage material stretch and flow, blank holders maintain the sheet in position while allowing controlled movement, and die radii influence the bending and unbending of the material. Accurate representation of these features ensures that the simulation can predict the forming process’s behaviour correctly, leading to better insights and optimisation of the manufacturing process.
Process Parameters:
Key parameters such as blank holder force, which controls the pressure on sheet metal to prevent wrinkling and ensure uniform material flow, must be carefully adjusted. Insufficient blank holder force can lead to undesirable wrinkling, whereas excessive force may cause tearing or excessive thinning of the material. Similarly, punch speed plays a critical role by influencing strain rates and material temperature during deformation. Higher punch speeds can increase strain rates and generate heat through friction, potentially altering material properties and affecting the forming process outcome.
Additionally, lubrication conditions are vital for reducing friction between the tool and the material, ensuring smooth material flow and minimising wear on tools. The type and amount of lubrication used significantly impact friction coefficients, which in turn affect forming forces and the overall quality of the final part. For processes involving thermal effects like hot forming, accurately setting temperature parameters is essential. The material’s response to deformation, including formability, strength, and springback behaviour, is directly influenced by temperature variations.
Springback Prediction:
Springback poses a significant challenge in sheet metal forming, where the material rebounds elastically after forming forces are removed, causing deviations from the intended shape. Accurately predicting and mitigating springback requires a multifaceted approach. Advanced computational methods such as finite element analysis (FEA) with sophisticated material models are crucial, accounting for complex factors like material anisotropy, the Bauschinger effect (where material softens after yielding), and residual stresses left in the material post-forming. Iterative simulations play a pivotal role in refining predictions by running multiple cycles to adjust tooling and process parameters iteratively, gradually achieving closer alignment with the desired part geometry. Engineers also employ compensation strategies such as over-bending or modifying tool geometry to counteract anticipated springback, which may involve altering punch and die shapes or adjusting the forming sequence to reduce residual stresses and improve dimensional accuracy. Moreover, detailed material characterisation through rigorous testing of stress-strain curves and hardening behaviours provides essential data for reliably predicting how the material will deform and recover during the forming process, ultimately ensuring more precise and successful sheet metal forming operations.
Incremental and one-step simulations:
The choice between incremental and one-step simulations in sheet metal forming hinges on balancing accuracy with computational efficiency. Incremental simulations adopt a step-by-step approach, dividing the forming process into discrete stages where the solver sequentially updates geometry and stress states. This method excels in capturing intermediate states crucial for accurately predicting complex phenomena like springback, wrinkling, and localised necking. Despite its accuracy, incremental simulations are computationally intensive due to frequent remeshing and re-solving challenges, especially in maintaining mesh quality and achieving convergence during large deformations or complex geometries.
Conversely, one-step simulations tackle the entire forming process in a single solve, leveraging implicit methods to update geometry and stress states without iterative steps. This approach offers efficiency gains by minimising computational time and handling large deformations robustly. However, it sacrifices some accuracy in intermediate states and may present challenges in precise springback prediction and explicit capturing of forming sequences. Engineers often opt for one-step simulations when computational resources are limited or for initial feasibility studies, while using incremental methods for detailed analysis where accuracy in intermediate steps is critical.
In practice, hybrid approaches may combine both methods: utilising one-step simulations for preliminary assessments and switching to incremental simulations for in-depth analysis of specific forming stages. Regardless of the approach chosen, validation against physical experiments remains crucial to ensure simulation results accurately reflect real-world outcomes, guiding effective design decisions and enhancing the reliability of sheet metal forming processes.
Solver Settings:
Solver settings play a pivotal role in simulation accuracy and efficiency. Implicit solvers excel in scenarios involving large deformations and nonlinear material behaviour, such as metal forming processes. These solvers use an iterative approach to solve the governing equations, allowing them to handle stiffness matrices effectively. While implicit solvers are robust in managing complex geometries and material behaviours, they typically require longer computation times due to their iterative nature. On the other hand, explicit solvers are suited for dynamic events like impact simulations, where they excel in handling transient phenomena and high strain rates. These solvers employ an explicit time-stepping scheme, simplifying the solution process but potentially encountering stability issues with large deformations.
Post Processing:
Post-processing analysis is essential for interpreting simulation results and refining manufacturing processes. It involves scrutinising formed parts for thinning and wrinkling, which can compromise part quality and manufacturability. Adjusting process parameters or die designs based on post-processing insights can mitigate these issues effectively. Identifying stress concentrations is also critical, as these areas are prone to failure or fracture. By pinpointing stress concentration points, engineers can implement design modifications, change materials, or incorporate stress-relieving processes to enhance part durability. Analysing the forming sequence provides valuable insights into how materials flow and deform throughout the process, helping detect unexpected defects or behaviours at intermediate stages. Lastly, post-processing involves identifying and addressing any unexpected features like undercuts or material thinning through process refinements or tooling adjustments, ensuring the manufacturing process meets quality standards and design specifications.
Key Takeaways:
Successful sheet metal forming simulations rely on meticulous consideration of several critical factors, from robust material behaviour modeling and precise solver settings to comprehensive post-processing analyses. With Dynaform, a sheet metal forming simulation tool designed to improve all phases of the die development process, engineers can accurately model material responses using advanced algorithms that account for complex behaviours like anisotropy and plasticity. Mesh quality and boundary conditions are carefully managed within Dynaform® to ensure simulations capture detailed physical phenomena accurately. Tooling geometry, process parameters, and effective springback prediction strategies further enhance simulation fidelity and guide optimised manufacturing processes. Whether opting for incremental or one-step simulations, Dynaform balances computational efficiency with accuracy, supported by thorough validation against physical experiments. By integrating these methodologies, engineers can confidently predict, refine, and enhance sheet metal forming processes, ultimately improving product quality and reliability in industrial applications.
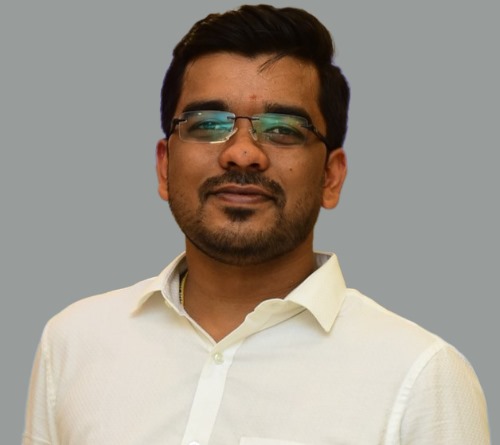
Akshay has completed Masters in Tool Design and has accumulated over a decade of experience in the field of stamping tooling while working with several major tool manufacturers. As a Product Manager for Dynaform, he focuses on enhancing Dynaform to meet evolving market demands, advance sheet metal technology, and exceed user expectations.
COMMENTS